In the early 2000s, physicist Peter Nordlander and a graduate student, Emil Prodan ’03, spent a year running complex quantum mechanical simulations on a supercomputer to systematically investigate how the optical resonance of gold-covered nanoparticles would change along with changes to their geometry — like the width of their core or thickness of their outer shell.
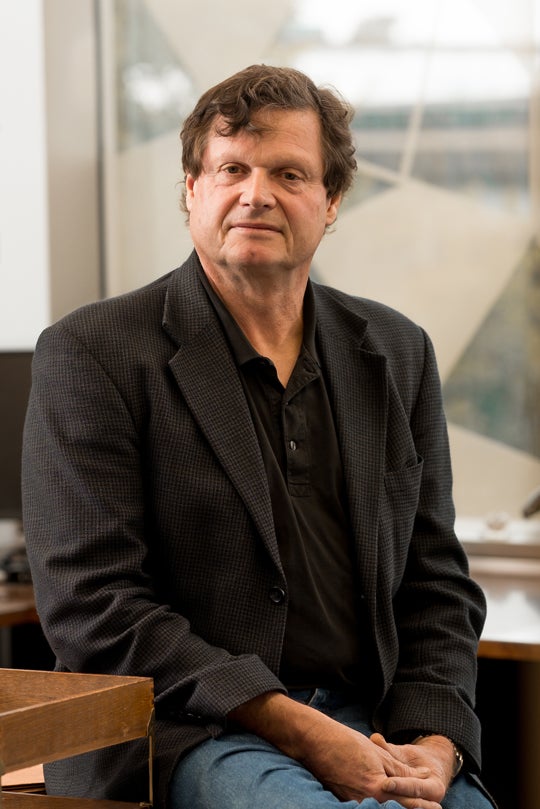
“These were giant calculations at the time,” Nordlander said, “fully quantum mechanical, many-body, theory calculations. As we got more and more results over a period of perhaps one year, we started to see some interesting, underlying, simple physics.”
The particles were gold nanoshells, which had been discovered at Rice a few years earlier in the lab of Naomi Halas, Nordlander’s longtime collaborator. Waves of electrons called plasmons slosh constantly across the metal surfaces of nanoshells. Plasmons respond to incoming light that matches their frequency, and as their oscillations change, so do their optical responses.
The phenomenon isn’t limited to nanoshells. “Anywhere you have conduction electrons — electrons that are free to move, like in a metal — you can have this plasmonic response,” Nordlander said. But the plasmonic response of nanoshells had an interesting feature that proved critical in his and Prodan’s development of a new theory that helped demystify plasmonics.
Like a sheet of paper or a piece of foil, a nanoshell’s gold covering has both an outer-facing and inner-facing side. Each side is a surface with its own plasmon, and the two plasmons interact. In looking at the numerical output of thousands of simulations of these interactions in nanoshells with differing geometry, Nordlander and Prodan saw something familiar and made an intuitive leap.
“We said, ‘It looks like the response is a hybridization.'” Nordlander recalled.
Published in Science in 2003, their plasmon hybridization theory mapped perfectly onto a concept in molecular orbital theory called orbital hybridization, which is taught in virtually all introductory chemistry courses.
“It provides a very quick, initial design of a plasmonic diagram structure,” Nordlander said. “You want to have a structure that can do something. You can just think about it in hybridization and see, ‘There’s going to be three particles there and two particles there, and so on.’
“It really, truly had impact,” Nordlander said. “Before plasmon hybridization, the only way to understand and calculate the optical response of plasmonic nanostructures was by doing massive, numerical modeling. And there was no way of understanding what would happen if you changed one part of the nanoparticle. For instance, ‘What happens if you have two particles and you move them closer together?’”
He said plasmon hybridization provides a quick answer to such questions. It’s also versatile, covering plasmonic interactions in all metallic and metal-dielectric nanostructures and patterned nanosurfaces, including metamaterials. That utility attracted newcomers, significantly broadening the scope of nanophotonics research.
Regarding potential similarities to the C60 discovery, Nordlander said, “I really think the common thread here is that they each enabled applications that are good for humanity. Nano-optics started out as something very fundamental. But as the field took off, it has really become clear that it has a tremendous application for society, whether it’s doing sustainable chemistry, making chemicals with very low energy, no greenhouse gasses, etc., or doing early detection of cancer or the cancer therapy that Naomi developed early on.
“If you look at any applications within the broad field of science, optics and nano-optics is really a growth field,” he said, adding that he feels fortunate to have co-authored such a widely used “theory for that very basic optical response of nanostructures.
“Let’s say it this way,” he said, “It's the right place to be, now and for the next 20 years.”