The Race for Stillness
In 1995, the world became captivated by an extraordinary scientific competition — a race where victory depended not on speed, but on achieving near-ultimate stillness. First predicted by Satyendra Bose and Albert Einstein in the 1920s, the creation of a Bose-Einstein condensate promised to unveil a rare quantum state where particles that have been cooled to near absolute zero merge into a single, unified “super-atom.” Physicists believed atoms in this state had never existed naturally anywhere in the universe, making its creation a true first of firsts. The pursuit alone was thrilling, but what truly set this race apart was how it defied conventional physics, bridging the microscopic world of quantum mechanics with observable, macroscopic effects. Top physics laboratories around the globe engaged in a high-stakes showdown to fulfill Bose and Einstein’s decades-old vision, each wanting to be the first to turn theory into reality.
The pursuit of this elusive quantum state was marked by a shared stillness, with experiments often occurring late at night when conditions were most stable for the sensitive equipment. On a humid July evening at Rice, the faint hum of machinery filled the room as three physicists studied the curious rings that had appeared on their imaging system. Led by physics professor Randall Hulet, the team — including graduate students Curtis Bradley ’97 and Cass Sackett ’99 — had worked for years toward a shared focus of the realization of a Bose-Einstein condensate. As they analyzed the unexpected patterns, it wasn’t immediately clear what they were seeing. Were these rings the long-awaited evidence of a transformative success or a quirk of an imaging system they were not yet completely familiar with?
The team’s finish line was rooted in one of quantum physics’ most fascinating ideas, a phenomenon that demanded a new way of thinking about matter. At the quantum level, particles aren’t just tiny points — they also act like waves. Instead of having fixed positions, their locations are “smeared out” into regions of probability described by mathematical functions known as wavefunctions. Imagine ripples spreading across the surface of a pond. At higher energies, the ripples are small, distinct and move quickly. As the energy decreases, however, the ripples slow down, expand and begin to overlap, eventually forming a single, unified wave. For particles, it works much in the same way — their wave-like nature depends on energy, with smaller wavelengths at higher energies keeping them distinct. In other words, wavelength is inversely proportional to momentum. At room temperature, the particles’ wavelengths are very small, but as temperature decreases, their motion slows and their wavelengths grow larger. At temperatures less than a millionth of a degree above absolute zero — just a whisper away from nature’s lowest temperature limit — these waves can overlap entirely, becoming one mathematically identical object. Such a state of quantum matter was purely theoretical when first proposed by Bose and Einstein, over 70 years prior, due to a lack of technology capable of achieving such extreme temperatures. Decades later, breakthroughs in laser cooling, evaporative cooling and magnetic trapping brought the theoretical within reach.
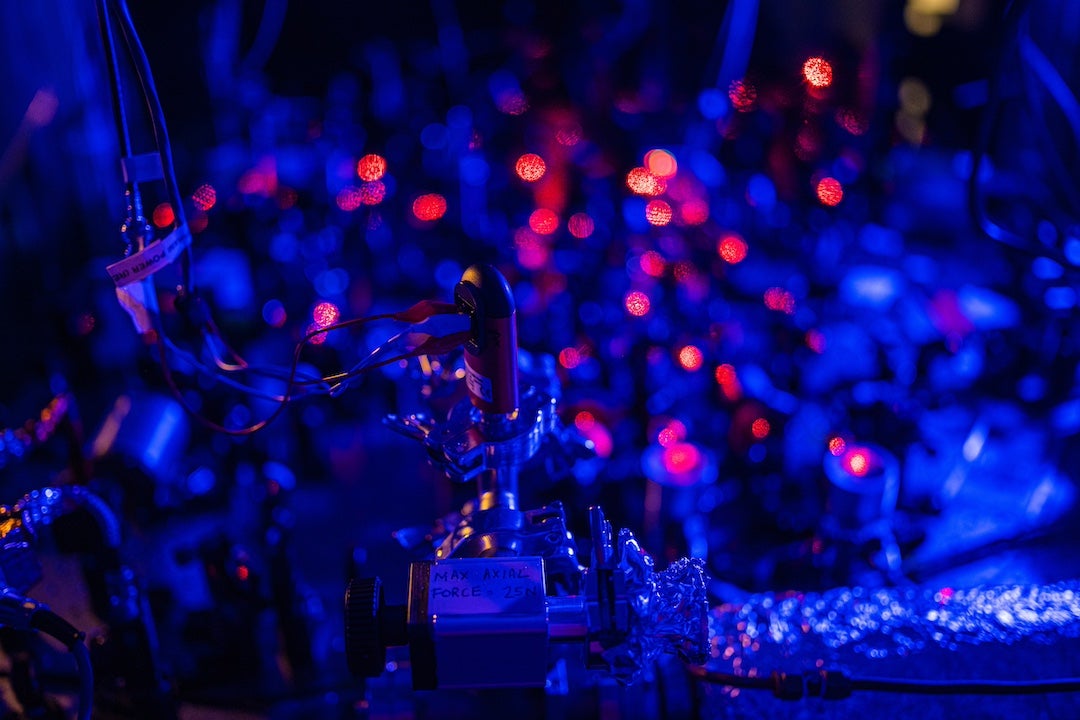
For Hulet, this transformative era was the starting point of his journey into the field. As a graduate student at MIT under Dan Kleppner, Hulet was familiar with spin-polarized hydrogen, a promising system at the cutting edge of low-temperature physics. “Dan had, for many years, been trying to see Bose-Einstein condensation of hydrogen, and many of the developments came out of his lab” Hulet recounted. “I got interested in laser cooling and atom trapping when I was at MIT.” This interest led Hulet to his postdoctoral work at the National Institute of Standards and Technology (NIST) in Boulder, Colorado, a hub of groundbreaking research in atomic, molecular and optical physics.
At the foundation of this field is a distinction between two fundamental classes of particles — bosons and fermions — that dictate the quantum behavior of matter. Every particle in the known universe falls into one of these categories, determined by spin, an intrinsic property akin to angular momentum, alongside mass and charge. Although often compared to a spinning ball, spin does not involve literal motion but reflects a fundamental characteristic of the behavior of a particle. Particles with integer spins, such as photons (spin-1), are bosons, while particles with half-integer spins, like electrons (spin-½), are fermions. When particles combine to form atoms, their total spin — determined by summing the spins of their constituent protons, neutrons and electrons — dictates whether the atom acts as a boson or fermion.
The wavefunctions of bosons, named after Satyendra Bose, can overlap at near-absolute zero, merging into one unified entity. Fermions, however, are restricted by the Pauli Exclusion Principle, which arises from their asymmetric wavefunctions and prevents them from occupying the same quantum state. This fundamental difference shapes everything from the structure of atomic orbitals, where electrons are limited to pairs with opposite spins (+½ and -½), to the unique behaviors observed in ultracold matter.
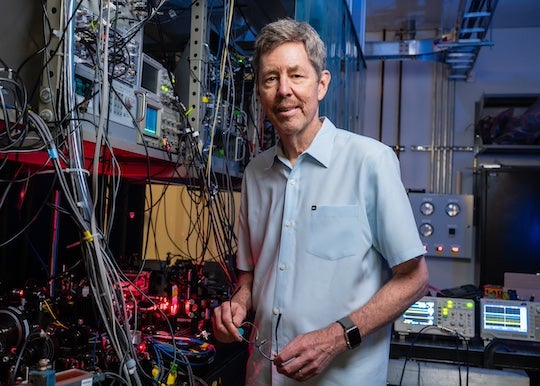
In 1987, when Hulet applied for a faculty position at Rice, his application contained a proposal for cooling lithium atoms to low enough temperatures to first create a Bose-Einstein condensate of lithium-7, bosons by nature, and then, to use similar methods to cool lithium-6, which are fermions. The success of Hulet’s lab hinged on the ingenuity of its tools and the collaborative dynamic of the team. Each member brought a unique contribution to the experiment, working together to create a system capable of cooling and trapping lithium atoms. Unlike the atoms used in other experiments of the time, lithium presented unique theoretical and experimental challenges that demanded persistence and creativity to overcome.
One of the key components of the experiment was the permanent magnet trap, intended to confine the atoms using a stable magnetic field. An earlier member of the team, Jeff Tollett ’95, designed and built the trap for his Ph.D. work. To cool the atoms, the team employed laser cooling, which slowed the atoms’ motion using carefully tuned laser beams, and evaporative cooling, which removed the hottest atoms to leave behind a colder, denser cloud. Laser cooling acts as a precision braking system, using beams of light to slow down the atoms, while evaporative cooling is like letting steam escape from a cup of hot coffee — removing the hottest atoms to leave behind a cooler, more concentrated group.
The imaging technique was another vital component of the experiment, allowing the visualization of the condensate. Although Bose-Einstein condensates are macroscopic systems, they are still incredibly small, low-density gasses requiring advanced imaging to detect. Bradley and Sackett adapted phase-contrast imaging, a method from biology, to reveal the condensate’s interference patterns. “Curtis and I proposed this imaging technique,” Sackett explained. “We sort of passed the ball back and forth a couple of times before he came up with something that really worked.” This technique allowed the team to detect subtle interference patterns generated by the condensate, an approach Hulet described as “entirely invented by them.” The team’s collaborative process extended beyond individual tools. “The experiment wouldn’t have happened without them,” Hulet said of his students, emphasizing how the group worked together to realize its goal.
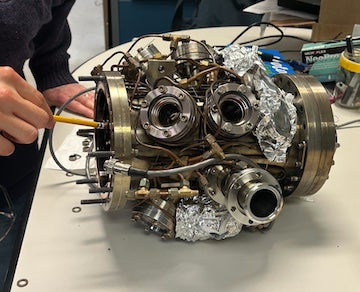
Using these techniques, the lab successfully cooled lithium-7 to 100–400 nanokelvin, or about -459° Fahrenheit—a temperature less than one millionth of a degree above absolute zero. To put this into perspective, the coldest observed temperature in the universe, about 1 kelvin, is in the Boomerang Nebula. This means Hulet's lab at Rice has contained, at multiple points in time, atoms that are 10 million times colder than the coldest known place in nature.
The excitement reached a fever pitch on June 5, 1995, when Eric Cornell and Carl Wieman observed the first Bose-Einstein condensate at JILA, a joint institute of the University of Colorado Boulder and NIST. Consisting of approximately 2,000 rubidium-87 atoms, the condensate measured about 20 micrometers in diameter — roughly one-fifth the thickness of a sheet of paper. About one month later on July 14, their findings were published in Science, solidifying their place in history and earning them a share of the 2001 Nobel Prize in Physics. The pressure was higher than ever for the Rice team, who worked tirelessly to push their experiments over the finish line.
Hulet’s choice of lithium-7 introduced an additional obstacle not faced by other teams, as lithium-7 atoms exhibit attractive interactions that naturally pull the atoms toward one another. Another team in Hulet’s group discovered this property in January 1995, six months before the JILA results were published. While intriguing, this attraction posed a serious challenge. Conventional theory predicted that such interactions would cause the condensate to collapse into a dense clump rather than forming a stable quantum state. Some physicists believed it could be possible to stabilize the system under carefully controlled conditions, while others remained skeptical. “There were quite a few people who thought that a condensate with attractive interactions was impossible,” Hulet reflected.
Despite the uncertainty, Hulet and his team pressed forward, hypothesizing that the quantum zero-point energy — the inherent energy present in all quantum systems no matter how cold they become — could stabilize the system if the number of atoms in the trap was carefully controlled. This would give rise to a condensate considerably smaller than those seen with the rubidium-87 atoms used by the JILA team. It was a risky approach, “We were close… we weren’t stopping,” Hulet said, “and so we just pushed forward.”
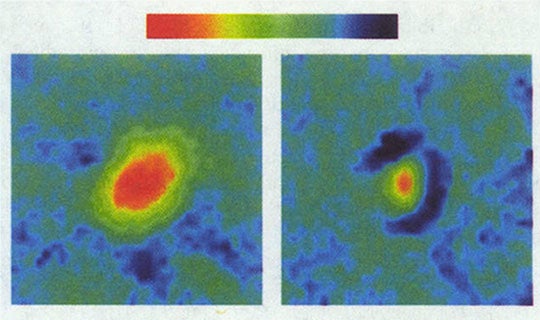
The breakthrough for the Rice team came on July 10, 1995, just about one month after JILA, when they observed interference rings that they believed indicated the presence of Bose-Einstein condensation in their lithium-7 system. Late that night, the team stared at the faint rings on their imaging system, uncertain whether they could definitively conclude that these rings were interference from the condensate. “It was not as good as it could have been because our results were not completely clear,” Sackett explained. “We saw these rings around it… a little faint, but as the atoms got colder, the rings got more pronounced.” The team later realized that the condensate was smaller than their imaging resolution, producing artifacts that complicated their interpretation. Even so, they concluded that the rings in their carefully collected data appeared in the places expected of a small Bose-Einstein condensate. “We were all elated for about five minutes, and we made sure that what we saw was repeatable,” Hulet later recalled. “And then 15 minutes later, we were all out of there because we had been working for 48 hours straight.”
The timing of Hulet’s discovery put his team in quite an unusual position. On July 14, the same day Science published JILA’s findings, a reporter from The New York Times contacted Hulet for his perspective on the field of Bose-Einstein condensate research to further contextualize their story on JILA’s breakthrough. Hulet’s discussion of their own observation with the reporter caused their results to appear in The New York Times before they could be published in a peer-reviewed journal — a highly unusual scenario. “It was hard not to say, ‘Oh yeah, we did it too,’” Sackett reflected. Their paper, submitted to Physical Review Letters on July 25 and later published on August 28, detailed the successful creation of a Bose-Einstein condensate in lithium-7 observed implicitly through interference rings — an achievement that overcame many experimental obstacles. A paper from a team at MIT on Bose-Einstein condensation of sodium-23 was published three months later.
The extraordinary nature of the lithium-7 condensate set Rice apart. The team had achieved what some had thought impossible by stabilizing the condensate with fewer atoms in the magnet trap — an estimated 650 lithium-7 atoms compared to JILA’s 2,000 rubidium-87 atoms. This redefined the boundaries of quantum physics, opening new doors to the study of systems with attractive interactions. “Attractive interactions enabled a new set of experiments with even more things that could be done,” Hulet explained. “Being able to see the effect of attractive interactions on the condensate for the first time was deeply satisfying and, I think, important in the field.”
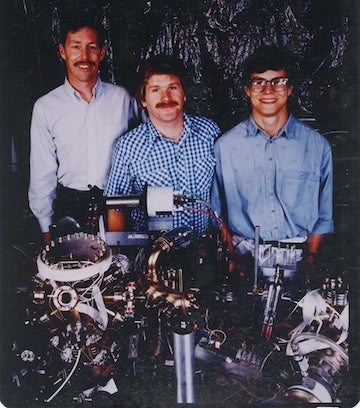
The success of this experiment was possible because of the collaborative efforts of Hulet and his graduate students. Jeff Tollett’s contribution to the permanent magnet trap, Curtis Bradley and Cass Sackett’s adaptation of phase-contrast imaging, and all members’ dedication are clearly reflected in their work, even 30 years later. Their creativity and technical expertise were instrumental in overcoming the unique challenges of working with lithium-7, laying the groundwork for a new era of experiments in ultracold quantum systems.
Hulet continues to work with lithium-7, its attractive interactions providing a basis for several of his later experiments. His lab has been pivotal in advancing our understanding of matter-wave solitons — stable, self-reinforcing wave packets that maintain their shape during propagation due to a balance between dispersion and attractive interactions. In 2002, his group created the first “soliton trains” in ultracold atomic matter, demonstrating that solitons do not diminish, spread out or change shape as they move. This achievement created new ways to study quantum mechanics in controlled settings, providing significant insights into the stability and manipulation of solitons. Hulet’s work has also explored higher-order solitons, known as “breathers,” that exhibit periodic oscillations in their amplitude, providing further insight into nonlinear quantum systems.
Despite the competition between JILA, Rice, and MIT, the broader story of Bose-Einstein condensation extends far beyond a single or even a few labs. It was the circulation of decades of shared knowledge, overlapping mentorships and collective innovation, resulting in an extraordinary moment where humanity peered into the quantum realm and found new possibilities.
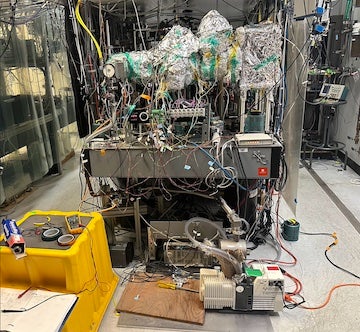
Today, Bose-Einstein condensates have expanded from a groundbreaking quantum discovery into tools that address some of the most complex challenges in modern physics and technology. The achievement of unified quantum-wave systems has revolutionized precision sensing, quantum simulations and wave phenomena studies. For example, atom interferometry can measure gravitational forces with unmatched precision. “It’s very sensitive to inertial forces,” explained Hulet, “making these systems a useful way of measuring things like gravity waves.” Such systems have applications in mapping gravitational anomalies, guiding spacecraft, and even detecting hidden underground structures. Sackett, who leads his own research lab related to Bose-Einstein condensation at the University of Virginia, elaborated, “You can make really good rotation sensors to keep an airplane or a spaceship going straight.” The applications continue to grow alongside the research, which has advanced significantly in the years since the initial pursuit of a Bose-Einstein condensate.
The story of the race for a Bose-Einstein condensate is not just about a breakthrough in physics, but also a testament to the curiosity and determination that drive humanity forward. For Hulet and his team, the journey was filled with challenges, late nights and the thrill of venturing into unknown territory, embodying the collaboration and perseverance of scientific discovery. Reflecting on this journey, Hulet’s advice to future scientists is simple: “Follow your love, follow your interest.” And in those words lies the heart of discovery — not just for physicists, but for anyone seeking to leave their mark on the world. The work at Rice is a celebration of what becomes possible when we allow our curiosity to guide us, proving that even in the coldest extremes of the universe, there is warmth in human determination and the pursuit of understanding.
-Magdalena Whelley ’27
This work was made possible with support from the Fondren Fellows program.